Unveiling the invisible: A short journey into fluorescence microscopy
Cell observation through high resolution images
Obsah
Fluorescence microscopy, a subset of light microscopy, leverages the fluorescence effect to visualize structures and processes at the nanometer scale. This technique relies on fluorochromes, which, when excited by specific wavelengths of light, emit light of a different color. The applications of fluorescence microscopy span various scientific fields, including biochemistry, biophysics, and medicine, enabling real-time observation of diverse cultures. The following blog post looks at the importance of fluorescence microscopy in life science applications and describes its general mode of operation.
Fluorescence microscopy - a subset of light microscopy
Fluorescence microscopy stands out as a captivating subset within the realm of light microscopy, offering unparalleled insights into the intricate world of cellular structures. By harnessing the phenomenon of fluorescence, this technique allows scientists and researchers to delve into the microscopic universe with exceptional precision. Unlike traditional light microscopy, fluorescence microscopy employs fluorochromes, molecules that emit light upon excitation by specific wavelengths. This not only enhances contrast and visibility but also enables the visualization of dynamic processes within living cells. In the exploration of cell observation through high-resolution images, fluorescence microscopy emerges as a powerful tool, unraveling the mysteries of cellular function and behavior at a level of detail previously unimaginable.
Optical precision for enhanced results
Components of a fluorescence microscope
Figure 1: Working principle of a fluorescence microscope
The visualization of fluorescence under the fluorescence microscope is ensured by special filters that allow the passage of individual wavelengths. Special filters of a fluorescence microscope include:
- Excitation filters
- Emission filters
- Dichroic beam splitter
Individual excitation filters allow the respective wavelength of light to pass which is necessary to excite a specific dye in the sample to be examined. The dichroic mirror reflects the stimulating wavelength to the objective, which concentrates the beam onto the specimen. The light reflected from the specimen is concentrated in the objective and in its excited state usually has a higher wavelength than the incident light. Passing through the dichroic mirror, the reflected light passes through the emission filter and is reduced to the wavelength of the emission. Residues of the stimulating light that have not yet been stopped at the dichroic mirror are filtered out at the emission filter. Ideally, only the emission light hits a detector built into the microscope and becomes visible in the respective color.
Optimum measurement results require uniform illumination, especially when a large field of view of several micrometers or millimeters is required. In the case of inhomogeneous illumination, for example, uneven activation of the molecules to be examined can occur. The result: The molecules in the center fluoresce more strongly than those in the periphery of the incident illumination beam (see Fig. 2). If the periphery is not illuminated equivalently to the center, shading continues to occur when the individually recorded image grids are later merged. Therefore, measurements such as cell and tissue samples cannot be used for reliable analysis.
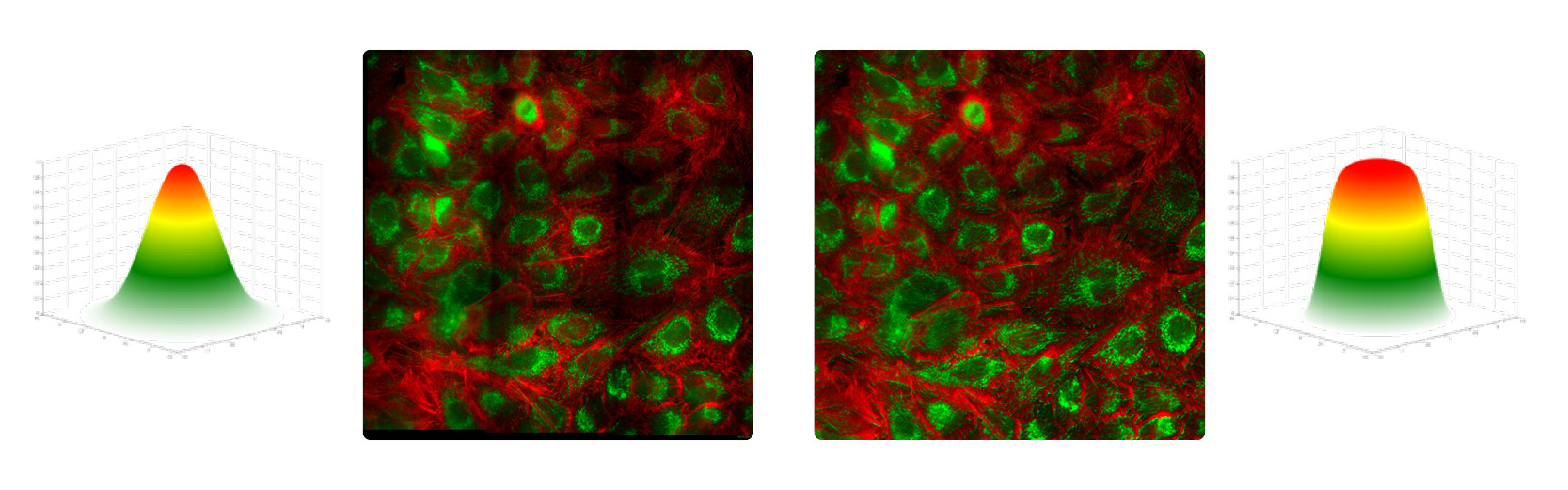
Problems like these can be overcome by using asphericons Top-Hat beam shaper the a|TopShape. This compact system utilizes aspheres for beam shaping. Its use enables the transformation of the Gaussian beam into a uniform Flat-Top profile and thus uniform illumination across the entire field of vision. The generated flat field illumination convinces by a high spatial coherence, unbeatable optical performance and a high homogeneity.
Fluorescence microscopy reference
As shown in the previous section, quantitative analyses within laser-based fluorescence microscopy can be complicated by non-uniform illuminations generated by Gaussian beam profiles. Factors such as light source and illumination optics affect the uniformity. These features are particularly challenging when a large field of view (FOV) is to be examined. Measuring images are generated in fluorescence microscopy by image grids. The individual images are acquired in such a way that the edges overlap and they can be assembled in post-processing. If the illumination is uneven, the final image will have darkened edges around each individual image - measurements of cell and tissue samples become unreliable. Another disadvantage of uneven illumination: uneven activation of molecules. Those closer to the center of the beam fluoresce more than those at the edge (see Fig. 2).
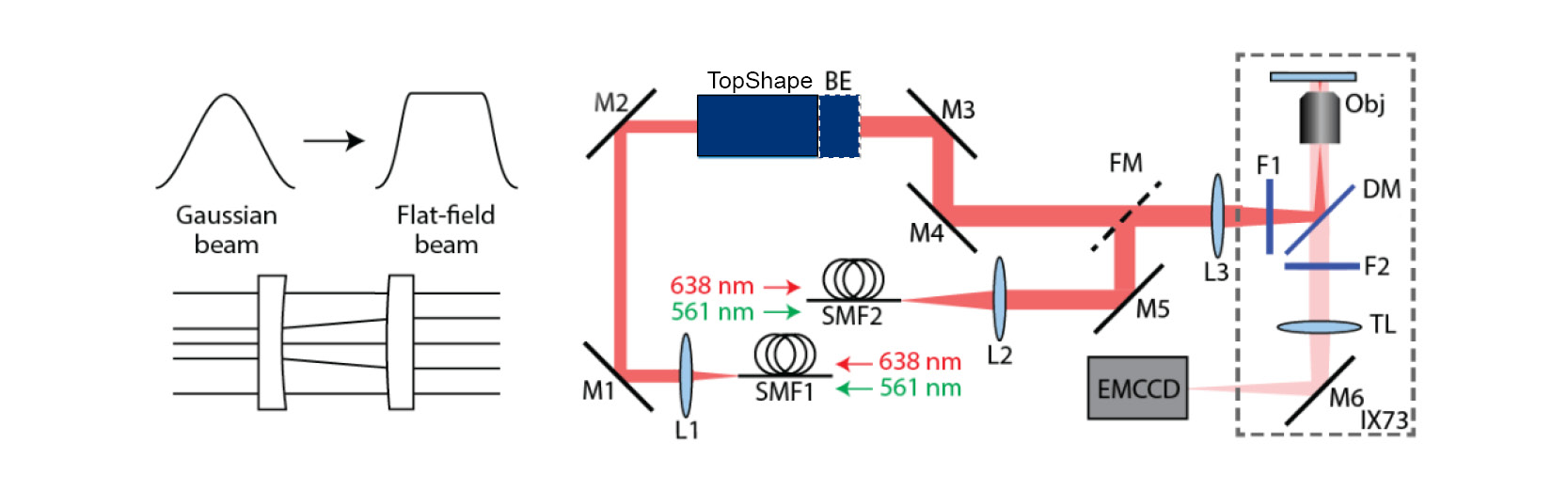
A research team at the College of Optics and Photonics, University of Central Florida in Orlando (CREOL), was able to overcome these problems by integrating aphericon’s beam shaper a|TopShape and the a|BeamExpander into a microscope set-up (see Fig. 3). The flat-field illumination (FFI) set-up shapes Gaussian beams into a uniform Flat-Top profile. The a|TopShape is extremely tolerant to variations in the size of incoming laser beams (± 10 %) and operates achromatically. The very good optical performance (homogeneity > 95%) enables uniform illumination and thus activation of the molecules. In addition, the FFI set-up enables borderless stitched imaging with minimal image overlap (5%).
For more information on the project, see our reference story.